Where should we look for life?
Developed in 1961 by the late Frank Drake, a giant of the SETI community, it was an initial attempt to ascertain how many intelligent civilisations might exist that humanity could potentially communicate with.
Every parameter was a best guess, nothing definitive was known, and whilst our knowledge has improved dramatically in the last 30 years – we are only marginally better off today and this improved knowledge only allows to confirm that planets exist around the majority of stars and that brown dwarves may also have planets that could, in theory, harbour environments conducive to life – back in 1961, Brown Dwarves were not even a thing at that point, they were theorised later in the 1960’s but the first unambiguous discovery was not made until the late 1990s.
In 1961 we assumed that most, if not all, other stars had planets, but we had no evidence to support this, our knowledge about the planets in our own solar system and the formation of life was so scant many felt that life would be found on Mars, even Venus was still considered to be potentially a living world like Earth – but a permanent steaming rain forest based on the cloud layers we could not see through and calculations implied an average surface temperature of around 35°C .
By the end of the 1962, thanks to Mariner 2, we knew Venus was a broiling hell hole not conducive to life, with layers of sulphuric acid in the upper atmosphere that fell as rain from one cloud layer to another. The data we received back indicated that the surface was many 100s°C, later confirmed to the be the hottest planet in the solar system with average daytime temperatures of 467°C, we now know the average surface pressure is more than 93 times that of Earth.
Mars, which had been visited by Mariner 4 in July 1965, destroyed the idea of a global civilisation existing on the surface, in fact, even the idea of seasonal vegetation was dismissed when Mars was shown to have surface temperatures well below freezing, its atmospheric pressure was similar to Earth’s at about 25 miles altitude and made of 95% Carbon Dioxide – it was a desiccated desert dryer than the driest places on Earth.
Graph 1 shows the highest and lowest points on the terrestrial planets.
Graph 1
Graph 2 shows the atmospheric pressure (Psi) for these points and the global average for the planet.
Graph 2
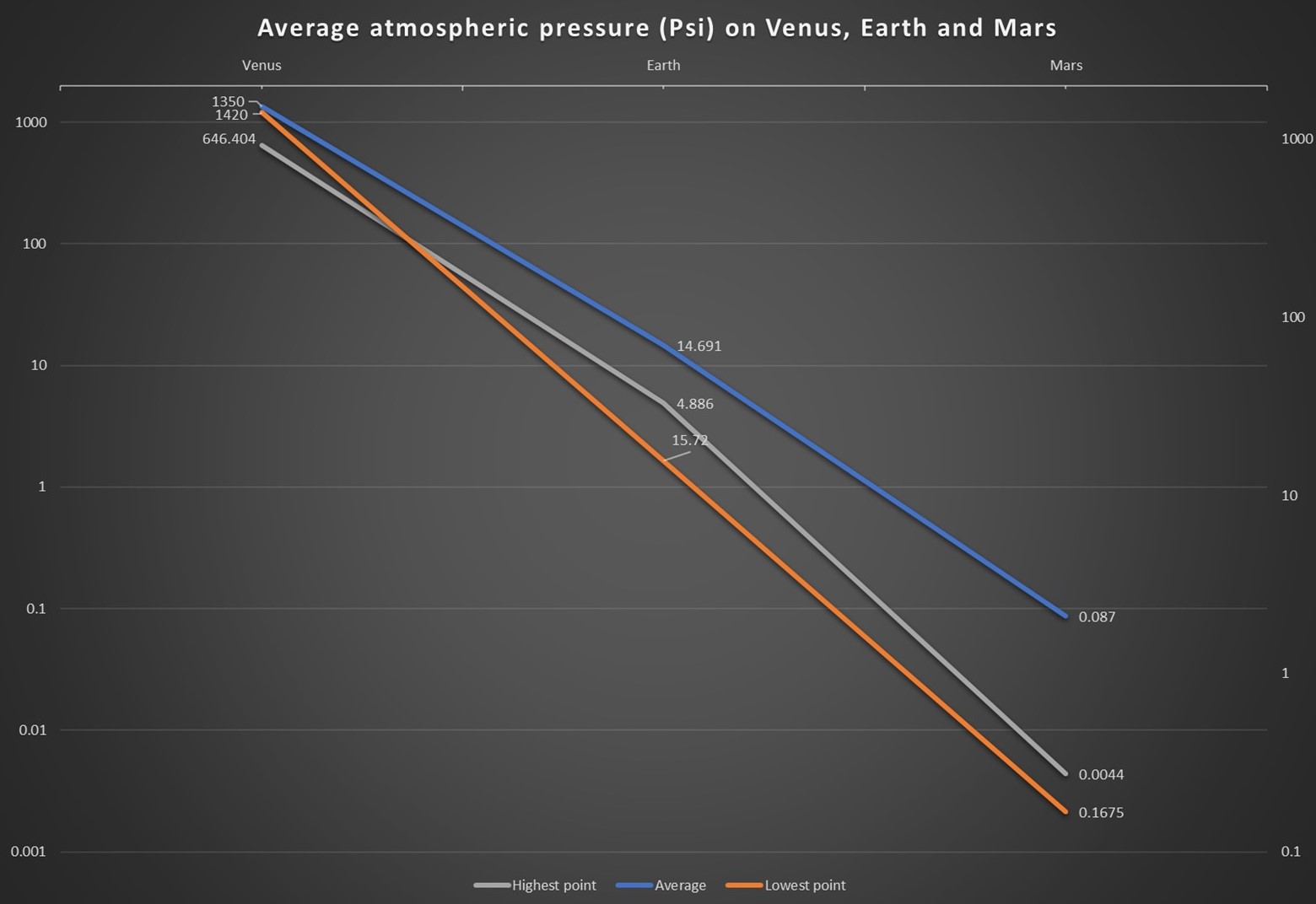
In those heady days of early exploration, it was concluded by many that there was no other place in the solar system that may have supported the development of life as we could understand it.
Over the decades our view of Mars has changed, it is now believed that that Mars may once have hosted a biomass as complex as early Earth. Mars lacks a global magnetic field to protect it from ionising radiation as particles stream out from the Sun, the “solar wind”, as such, the atmosphere has been slowly ablated and reduced, resulting in the loss of gas pressure, temperature and also any bodies of water on the surface. It is currently believed that as Mars’ surface environment evolved and changed, so life, unable to cope with the dramatic changes, died out, possibly as much as 2 billion years ago.
There is a hope that life may have survived longer, below the surface and possibly in layers of permafrost near to the two polar ice caps, in fact, some hope that life still exists below the surface today where conditions are conducive. We know Mars has a hot interior, it may not produce a global magnetic field, but it is not fully crystalline just yet, heat still migrates to the surface, in fact, there is circumstantial evidence for volcanism in the recent past (a few tens of a millions of years), this means that there could be a layer below the surface where hydrated minerals are warm enough to sustain a biomass which uses chemical reactions to support biological processes instead of photosynthesis, as plants and many bacterial species do on Earth.
In the last decade we have discovered a biomass below the surface of Earth that may contain up to 100x the carbon mass of all life on the surface of the planet, so looking for life below the surface of a world makes a lot of sense, where it would be protected from the harsh environment that may exist at the surface.
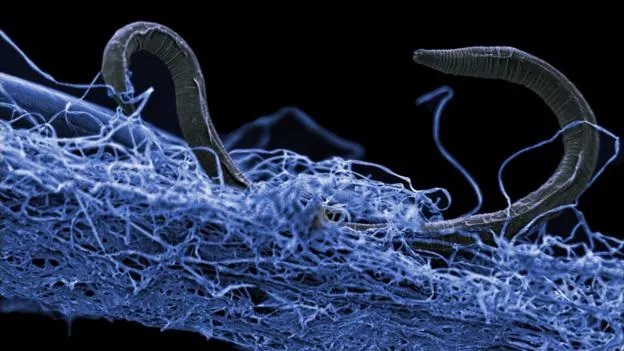
However, in recent years there has been a revolution in our understanding of the outer solar system, thanks to the Dawn mission we now know that Ceres is not an asteroid, at least not one as most people think about them, but a dwarf planet, but it is not a dead world. Dawn has shown that it has a new form of volcanism, a relatively young surface and gravimetric, as well as other data, suggests strongly that it has a warm rocky interior and likely a slushy, salty global ocean below a relatively thin silicate and ice frozen surface. Ceres is around 30% the mass of the inner solar system asteroids, but it is still 14 times less massive than Pluto, it has no known magnetic field or atmosphere.
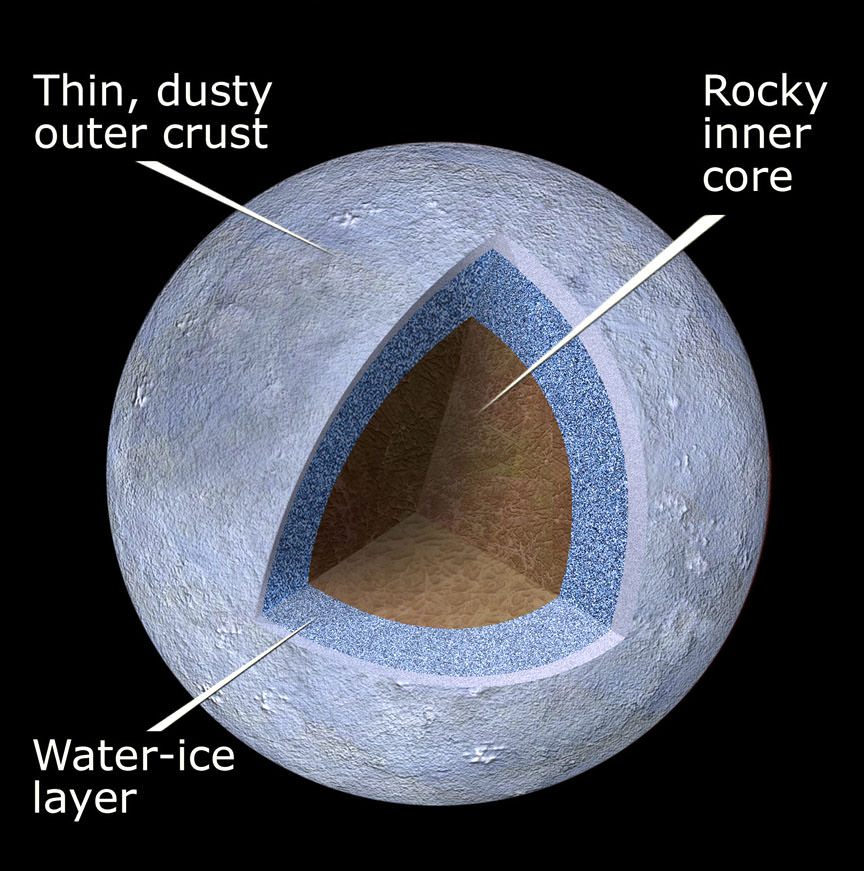
As we move out of the inner solar system, we have three of the planet sized moons of Jupiter, Europa, Ganymede and Callisto, which show strong evidence of subsurface global oceans, with Europa erupting water vapour, minerals and even organics into space from its fractured frozen surface.
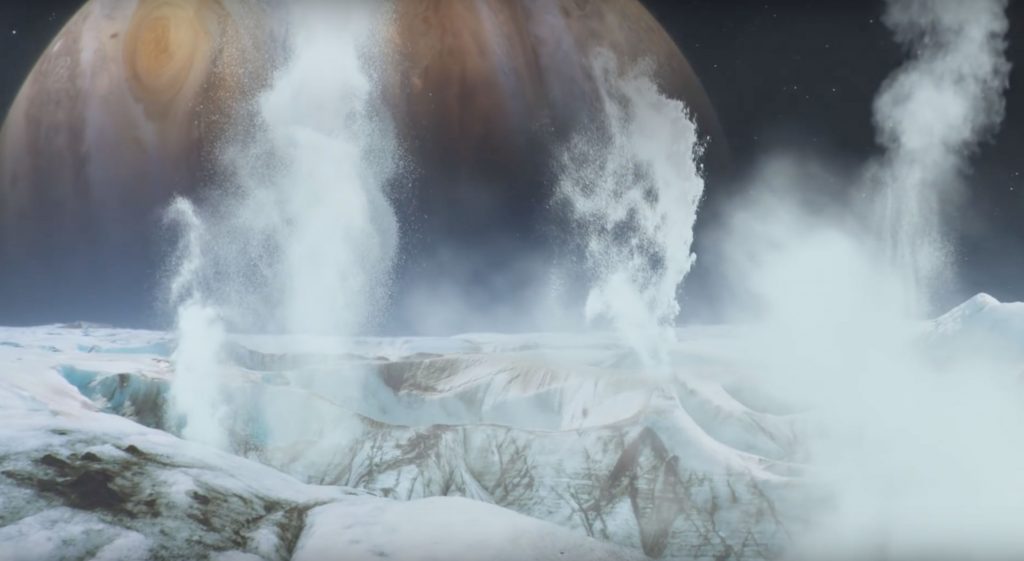
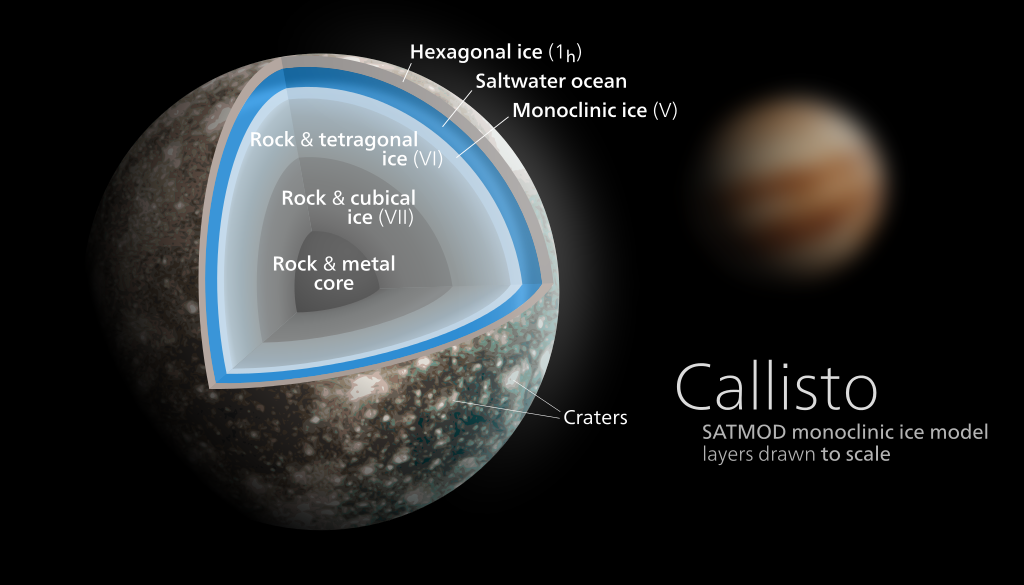
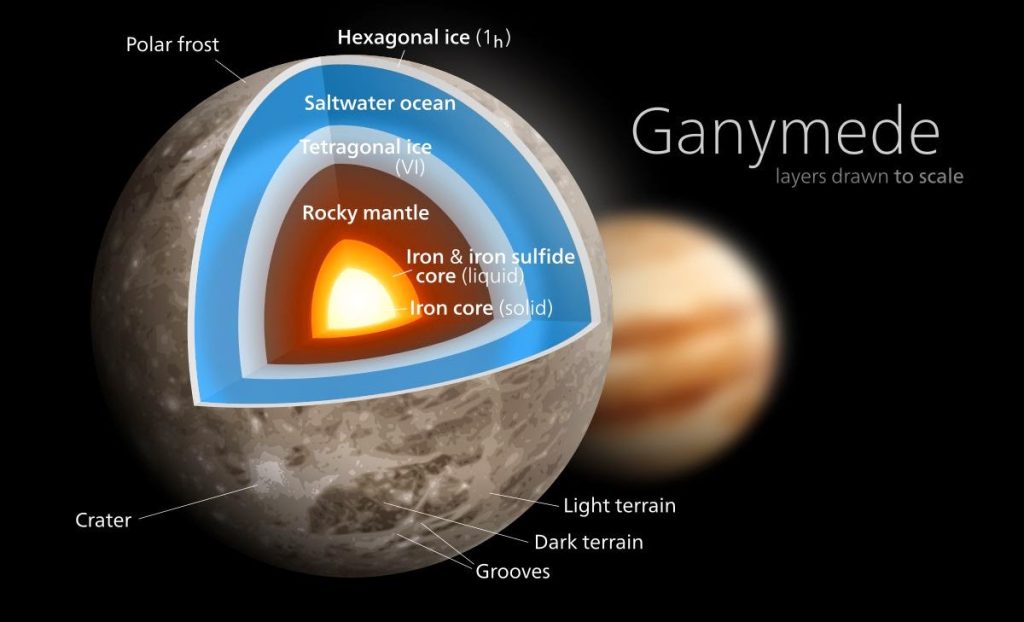
The intriguing thought process is that Europa could have some form of biological processes going on in that ocean and it may even have life, Callisto may have a warm interior due to gravitational interactions with Jupiter and it’s fellow giant Moons, but we know that Ganymede has solid and liquid cores, helping to generate a weak global magnetic field – but even Enceladus, a dwarf planet sized moon of Saturn also appears to have a subsurface ocean, as does Titan, we already were aware that this intriguing and mighty moon (second only to Ganymede), a planet by any other descriptor, has a complex atmosphere and surface environment, with lakes, rivers and even ocean basins (none of which are water, but rather methane and ethane). Titan has a molecular Nitrogen (N²) based atmosphere almost 1.5 times the density of Earth’s atmosphere. The surface is rich in organic molecules and both methane and ethane act like water does on Earth. Many researchers have wondered if life could exist on the surface of this intriguing solar system body. In 2026, NASA will launch the Dragonfly mission to Titan, arriving in 2034, where it will spend almost 3 years flying around the moon gathering images and data.
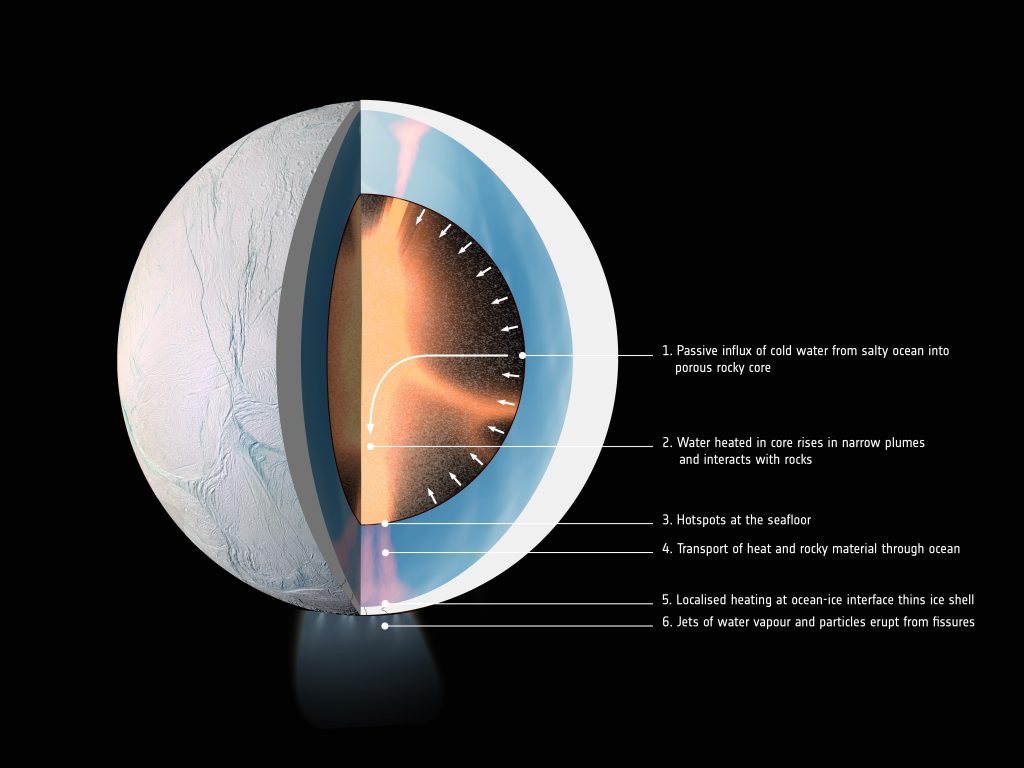
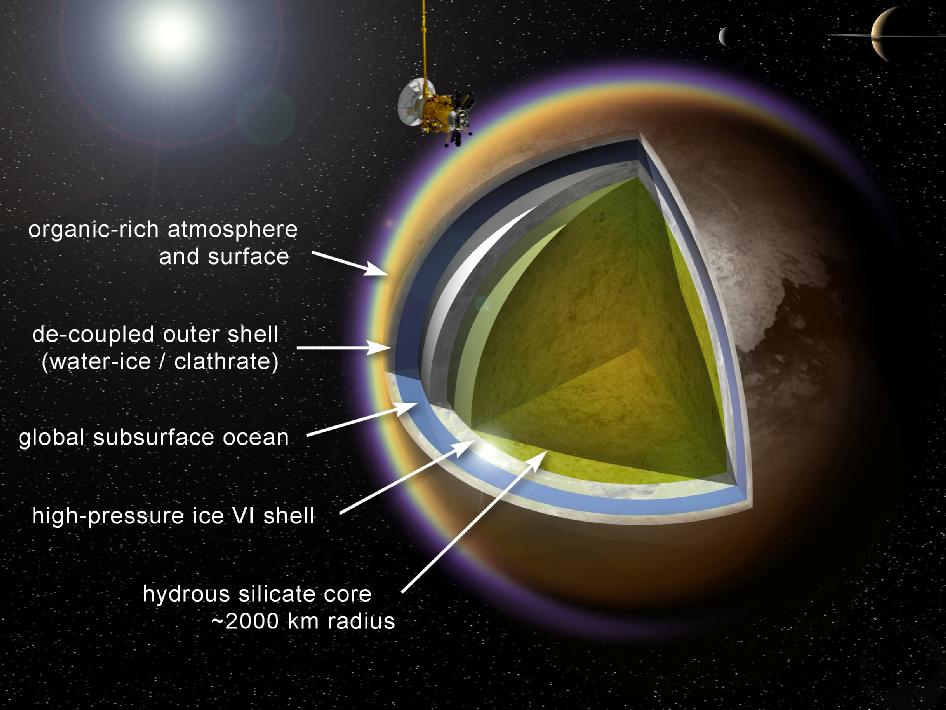
Neptune’s mighty Triton, is known to be volcanically active, and is most likely a captured Dwarf planet due to its retrograde orbit, is composed of up to 35% water by density, a composition which most resembles Pluto as far as we know, and potentially has a sub-surface ocean that will be heated from inside – if it contains organic material, like many of the larger bodies in the solar system seem too, then it is not impossible for life to have arisen in the depths of the global ocean. Even Pluto, demoted from planet status by the IAU in 2006, may have a subsurface global ocean, again, if there are organics in the mix, then could life of begun in this ocean?
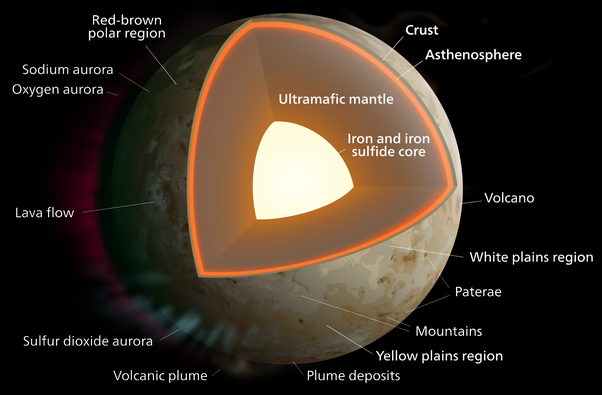
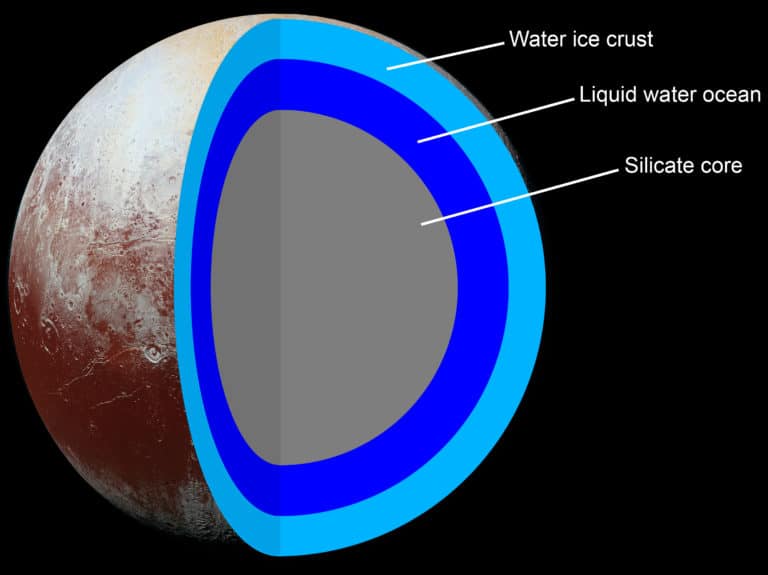
Do other bodies in the outer solar system, the so-called scattered disc and the Kuiper belt, also possess enough heat to maintain subsurface oceans – a few of these bodies are at least as large as Pluto, such as Eris, and the latest research on this dwarf planet, some 27% more massive than Pluto but marginally smaller overall, which is known to have an active, methane covered surface, may have a liquid salty ocean below the frozen crust.
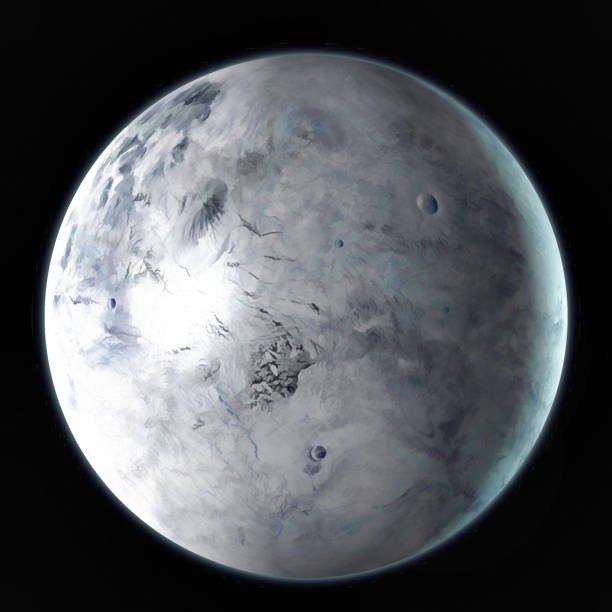
What does all this mean, well, perhaps our views of where life may arise are wide of the mark, it has always been assumed that a planet, or a moon, needed to be in the so-called habitable zone of their star, this is the region around the star where water can exist as a gas, liquid or a solid. This zone is different around different stars, diminutive Red Dwarf stars have very small but close in habitable zones, but as stars get hotter, the habitable zone will move out from the star and increase in depth. See below.
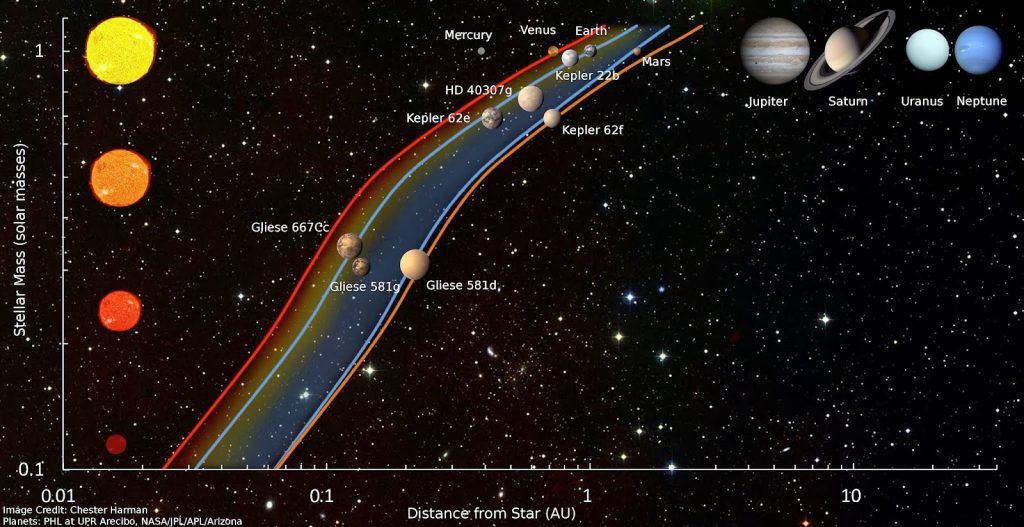
The habitable zone of the Sun starts just outside the orbit of Venus and stretches to just outside the orbit of Mars, toward the inner edge of the asteroid belt.
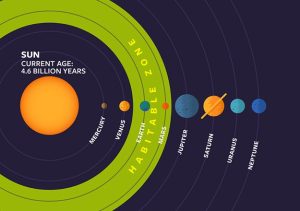
Venus would always be too hot where it orbits (107.9 million km) the Sun, water ice could not exist on the surface except in places that where it was permanently shaded from the Sun and was able to sustain sub-zero temperatures. Even if Venus had an atmosphere similar to Earth, the surface temperature would be around 35°C, so whilst liquid water could exist, water Ice would be unlikely -sorry skiers.
Mars (220.7 million km) is inside the outer edge of the habitable zone, but its thin atmosphere means it cannot retain the heat it receives from the Sun. Earth (149.6 million km), well, we happen to be about 30% of the way through the Sun’s habitable zone, so Earth is perfectly placed, from our perspective, for life as we know it. For comparison, if Mars were more Earth like in mass (this would allow a magnetic field perhaps and denser atmosphere) and its atmosphere was similar to Earth, the average surface temperature would be around 2°C, with the polar regions still being around -100°C and much of the surface would be locked in a perpetual ice age.
In recent years the habitable zone has been recognised as not the only place around a star where life may evolve, the so called “snow zone”, also referred to as the ice zone, could be conducive to the evolution of life it there are subsurface global oceans sustained by heat from within in the world in question, such as already mentioned exist at Europa, Ganymede and Enceladus to name a few.
This region sits outside the habitable zone, and as its name implies, ices and snows dominate on the surface of smaller worlds, this is where volatile compounds such as water, ammonia, methane, carbon dioxide, and carbon monoxide condense into solid ice grains. It was long thought that no life could exist outside the habitable zone due to temperatures, but with the knowledge we now have regarding internal heating mechanisms and salty global oceans below the surface, it is not inconceivable that life could have begun
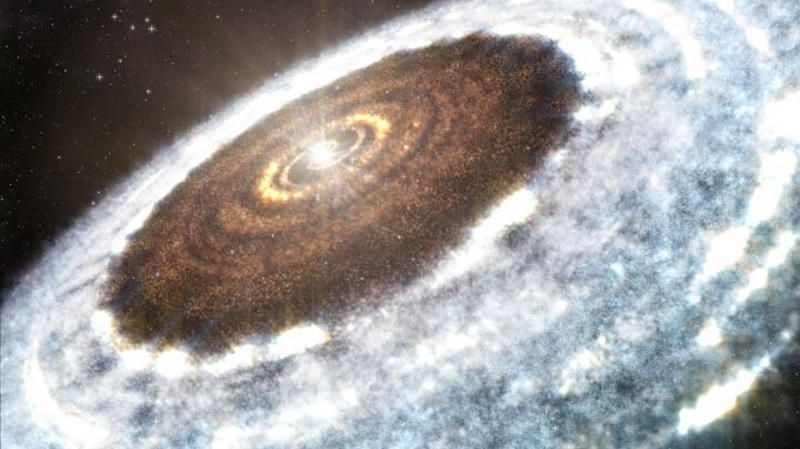
What the above informs us is that the early assumptions about where we may discover life were potentially naïve, but this is still partly the case today, until we can demonstrate that such environments can be the genesis of life, then it is as much speculation as it was decades ago, but at least we have a better idea of where to look.
Upcoming missions to Mars hope to look for life below the surface, there is still speculation about the controversy surrounding the results of the Viking missions in 1976, and current Mars systems are attempting to identify locations where life on the surface may have existed in the distant past, such as the ongoing investigations in Gale crater, but we also now have Europa clipper, a mission dedicated to the giant moon Europa, with teams even investigating how to, potentially, drop an autonomous submarine through the ice covered surface and into the ocean below, related to this are plans to design and hopefully send missions to Titan – the most interesting and intriguing body in the solar system.
But where does this take us with respect to the Drake equation, well, it shows we need to re-evaluate how we look at worlds, moons and life itself.
We, incorrectly in my humble opinion, assume intelligence means technology, but if we look at Earth, we have hundreds of intelligent species alive today if you broaden your definition to include complex language, tool use, social support behaviour and the ability to adapt, quickly, to changing environmental conditions.
Obviously, we can only communicate with a species that has technological communications systems that allow for long range communication across the void of space – in reality it would not be a conversation, but simply the receipt of signals that could not be natural and only created by artificial means, but this view may soon be overshadowed by our ability to detect chemicals in a planet’s atmosphere that could not be there naturally, or could not be maintained by natural processes, such as the industrial chemicals that humans pump into our atmosphere, many of which do not occur naturally or could not be maintained in the atmosphere by natural processes. Henry W. Lin et al 2014 ApJL 792 L7 wrote a paper, Detecting Industrial Pollution in the Atmospheres of Earth-Like Planets, on this very subject, and the hope is that now JWST is doing the science it was designed to deliver, we can use it to detect such things as tetrafluoromethane (CF4) and trichlorofluoromethane (CCl3F), which are the easiest to detect chlorofluorocarbons (CFCs) which do not occur naturally and only exist in our atmosphere because industrial processes produced and released them -assuming aliens are as bad at cleaning up their mess as we are of course.
Old SETI assumes that the intelligent life has developed to a technological level that would allow them or us to detect the radio leakage of our society, or perhaps deliberately beamed attempts at communication – the latter seems unlikely unless they already know we are here as it would simply be a waste of resources.
However, current understanding and thinking shows we can detect the chemical signature of an atmosphere, thus it is possible that we could detect an atmosphere with evidence of life within it – perhaps the chemical signature of chlorophyll, a paper on this was published by Timothy Brandt and David Spiegel on the this very subject, (Brandt TD, Spiegel DS. Prospects for detecting oxygen, water, and chlorophyll on an exo-Earth. Proc Natl Acad Sci U S A. 2014 Sep 16;111(37):13278-83. doi: 10.1073/pnas.1407296111. Epub 2014 Sep 2. PMID: 25197095; PMCID: PMC4169953.) which would indicate a living world. Back in 1993, in one of the last papers published by Carl Sagan, he and colleagues, published a paper in Nature in which they detailed how the Galileo mission had detected evidence of life on Earth as it flew by the planet on its way to Jupiter. Sagan, C., Thompson, W., Carlson, R. et al. A search for life on Earth from the Galileo spacecraft. Nature 365, 715–721 (1993) but perhaps we could detect semi-industrial chemicals in the atmosphere – which would indicate an intelligent species that had not yet reached a level of technology affording them radio communications, or perhaps the technology was a nascent one for them, similar to Earth at the start of the 20th Century.
The Drake Equation
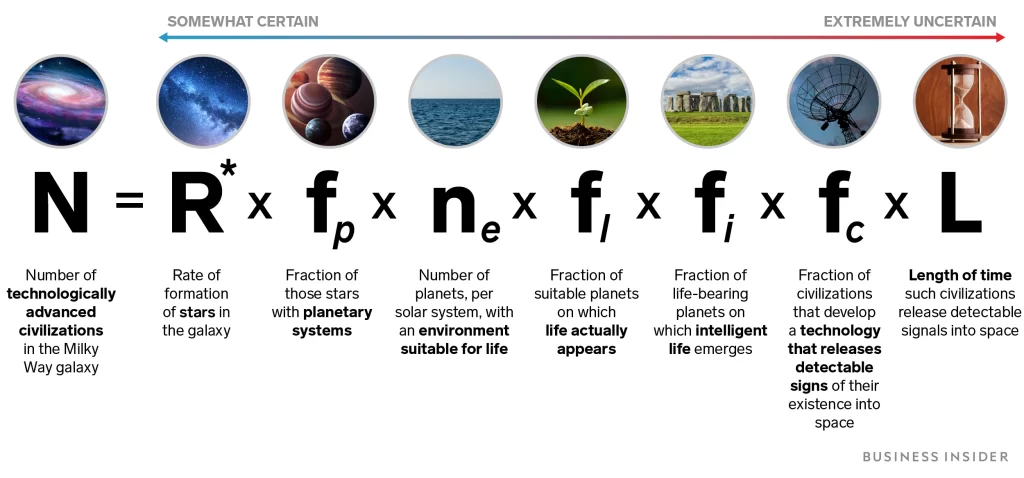
In the past, Drake equation calculations were based on the total stellar population of the galaxy, but we know that this line of thought is flawed, the galaxy is made up of a disparate population of stars of all ages, but the oldest, oddly known as population II stars, are very metal poor, these are the stars that formed in the early days of the galaxy, when Hydrogen and Helium were even more dominant than today, these stars contain vanishingly small quantities of heavier elements, referred to as metals, and it is highly probable that such stars will have metal poor planets, they may not even have terrestrial planets as we know them, and in these instances, life may not be able to arise and even if it does, without metals it is hard to see how it could develop technology. In those early days, the stars that had a mass much larger than the Sun have now reached the end of their lives, either throwing the by-products (metals) of their lives out into the galaxy as a Supernova, for stars <10 solar masses, or as the materials forming planetary nebula for stars >10 solar masses, however, dwarf stars, those less than 50% the mass of the Sun, are still happily burning hydrogen as stable stars, and many red dwarves will do so for trillions of years, so whilst we have a population of Population II stars in and around the galaxy, most are diminutive red dwarves, and it is unlikely they would have planets with the resources to create complex life.
We should look at where the stars are, in the galaxy, that we call population I stars, these are the stars that formed from the remnants of the first giant stars that erupted as supernova, seeding the galaxy will all the chemical elements we call metals, and thus are likely to have terrestrial planets as know them and any intelligent species has the capacity to then use these chemical elements in their technology, as humanity does.
The distribution of Population I and Population II stars in the Milky Way.
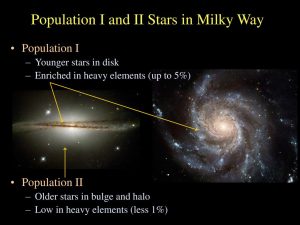
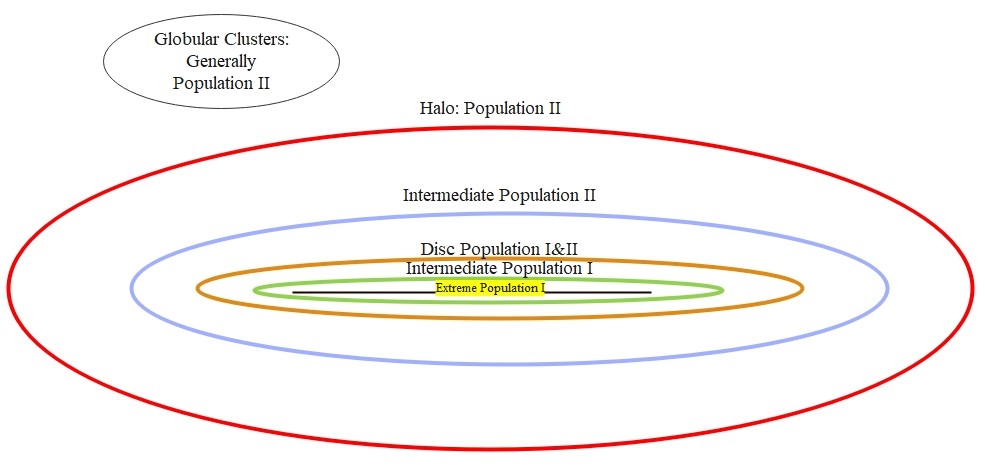
Our knowledge of the distribution and motion of the stars in the galaxy has increased exponentially thanks to the European Space Agency satellite, Gaia, which has been accurately mapping billions of stars since its launch to the Sun-Earth L2 point in mid-December 2013.
It is highly unlikely that many, if any, of the Population II red dwarf stars that exist possess life bearing worlds, and for ease we should concentrate where the highest probability exists for life bearing worlds, that is amongst the Population I stars.
So, if we restrict our search for life bearing worlds to those that orbit Population I stars, we need to restrict the number of stars we put into any equation for estimating the number of contactable civilisations.
Estimates are that around 42% of stars in our galaxy are population I stars, but how do we get to this number, let’s look at the estimates for the stellar population of the galaxy as a whole. Based on the latest research, let’s use the data in the table below – please note these are best estimates based on detailed research but likely hold inaccuracies.
Percentage of total | Total | |
Mass of the Galaxy (Solar Masses) | 100.0000000000% | 1,540,000,000,000 |
Stellar Population of the Galaxy | 16.2337662338% | 250,000,000,000 |
Red Dwarf Population | 85.0000000000% | 212,500,000,000 |
Dwarf Population | 10.0000000000% | 25,000,000,000 |
Sub Giant Population | 2.0000000000% | 5,000,000,000 |
Giant Population | 2.0000000000% | 5,000,000,000 |
Supergiant Population | 0.0900000000% | 225,000,000 |
Hypergiant Population | 0.0000001000% | 250 |
A Hertzsprung-Russell Diagram showing stellar groupings.
Now let’s look at this in more detail, how long do stars of a given type live, because this will surely impact if life and by consequence, a technical civilisation will have sufficient evolutionary time.
Stellar Mass | approx Life (yrs) | Average Spectral Class |
60 | 3 million | O3 |
30 | 11 million | O7 |
10 | 32 million | B4 |
3 | 370 million | A5 |
1.5 | 3 billion | F5 |
1 | 10 billion | G2 (Same at the Sun) |
0.1 | <1000 billion | M7 |
0.001 | <10,000 billion | M1 |
When we look at stars that are most likely to host high metallicity worlds where complex life and a technological society may arise, we need to rule out any that will not fit the bill for various reasons. Stars from Spectral A5 upwards are unlikely to host technical civilisations, not only are these stars highly energetic, but they are short lived, barely giving sufficient time for planets to evolve life in a stable environment, even should such an environment exist. Stars that are too small, those less than 20% of the Sun’s mass, whilst they live an exceptionally long time, any planet in orbit would need to be close in to have a stable climate, and that likely means it would be tidally locked, only showing one hemisphere to the host star – stars in this class are also often subject to huge coronal mass ejections, tens to hundreds of times the power of those from the Sun, which would bombard any planet in range with deadly radiation, likely stripping any atmosphere over time or at least making it untenable for life.
So where does this leave us, well, if we assume that for a stable environment to exist on a planet long enough for life to develop and then evolve, we likely need several billion years as a minimum, so we are looking at stars in the mass range of 0.5 to 1.5 times that of the Sun as that gives any planet from 3 billion years to hundreds of billions of years to develop.
How does this impact the number stars we should look at for evidence of life?
So, we rule out all Population II stars and we restrict our search for Population I stars in the mass range 0.5 to 1.5 solar masses that exist in a donut shaped section of the milky way whose inner edge is some 15,000 light years from the core of the galaxy and whose outer edge is some 30,000 we need to calculate the volume of space this encompasses.
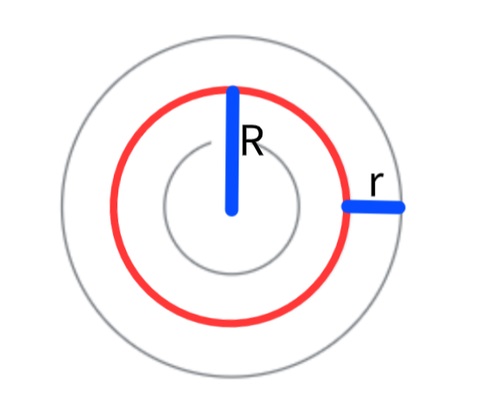
V=(πr2)(2πR)
Thus;
Centre of galaxy to the outer edge = 50,000 light years, thus the centre line of our donut, major axis (R), is 25,000 light years from the centre, and the minor axis is also 25,000 light years from the centre line.
Minor axis from centre to the axis (r) = 25,000
Major axis from centre to axis (R) = 25,000
so:
(r) = π x 25,0002= 3.142x 625,000,000 = 1,963,750,000
(R) = 2π x 25000 = 6.284 x 25,000 = 157,100
Thus: 706,960,000 x 94,260 = 308,505,125,000,0003 light years
Now we know the volume of space that is most likely to contain stars that are most likely to host life bearing planets – it’s big, very big, but the number of stars is also big, how big, well let’s take the information from the above tables and calculate it.
The galaxy contains around 212,500,000,000 Red dwarf stars, if we rule out 70% of these as being too low in mass or being part of the Population II group, then we have 63,750,000,000 stars, now we include the estimate for orange/yellow dwarf stars, that will be all those stars from around 0.8 to 1.5 solar masses, and this is estimated at 25,000,000,000, there are no Population II stars in this group as they have all reached the end of their age now or they have evolved onto the asymptotic red giant branch.
This gives us a potential population of 88,750,000,000 stars, not an inconsiderable number.
Now if we divide the potential volume of space by this number of stars
308,505,125,000,0003 light years / 88,750,000,000 stars = 3,476.114083 light years for each star – what is the diameter of this volume of space?
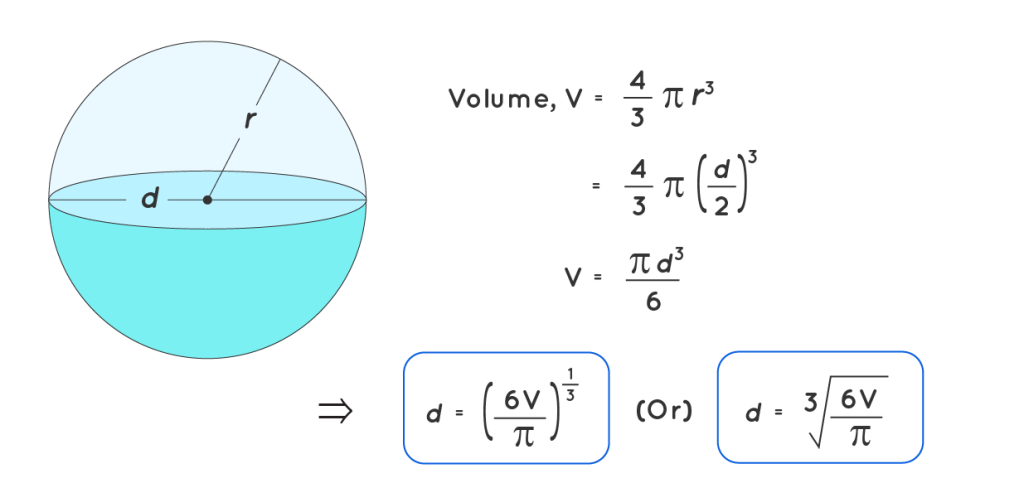
So, we have a sphere of space with a volume of 3,476.114083 light years which has a diameter of 244.4222 light years.
Thus, on average, life bearing worlds, in theory, should be an average of 244 light years apart, but we know this figure cannot be overtly accurate because we,
- Do not know the number of those stars with planets
- Do not know the number of planets those stars have
- Do not know how many of those planets will be in habitable zones
- Do not know how many develop life
- Do not know how many will develop complex life
- Do not know how many will develop intelligent life
- Do not know how many will develop technologically capable life
We are still in the realms of guess work, no matter how we look at things, so now we need to address the above points.
- What is the number of those stars with planets? Given what we are finding out from missions like Kepler, TESS and ground-based searches, it is highly likely that most, if not all stars, have planets, thus let’s assume that 98% of stars in our mass range have planets.
- What is the number of planets those stars have? This is a hard one, multi-planet systems are being found, but the types of searches being carried out to date are biased toward massive planets or planets in very tight orbits, but let’s assume that our solar system is average and state that the average star we should look at has 7 planets.
- What is the proportion of those planets in habitable zones of their star? Our solar system has 2 or 3 of its 8 planets in the habitable zone (depending on how you look at things), and the famous Trappist 1 system has three planets in its habitable zone.
Trappist 1 and Solar System Habitable Zone planets Based on this small sample, if we assume that 20% of the planets of these stars have planets in their habitable zone that gives us a number to work with.
- How many develop life? This is the first really hard guesstimate with no statistical basis, just rough guessing, but let’s assume that of those planets in the habitable zone, 1/3 (33%) will develop life it gives us a figure to start with.
Did life on Earth begin in the chemical and thermal soup that is found around hydrothermal vents at the bottom of the global ocean? Will we find evidence for life around similar systems in the oceans of other worlds? - How many will develop complex life? This will be, like 4 above, a best guess based on no statistics at all, but where life develops, let’s assume that 50% will develop complex life forms.
- How many will develop intelligent life? This is an easier one to estimate if we broaden our horizons as what constitutes intelligent life. Generally, most people, take humans as intelligent life and everything else less than that, but if we look at nature, look at how animals behave and how they adapt to changes of circumstances, we can say that intelligent life is a by-product of the development of complex life, we have hundreds, possibly thousands of species on Earth that could be classified as intelligent, thus, this figure can justifiably be 100% of planets with complex life evolve intelligent life.
- How many will develop technologically capable life? This is where we get into difficulty, we only have ourselves as an example, we have no evidence that a technical civilisation has existed before on Earth or any other world, but we do know that human civilisations have existed in the past, and not all developed technology, although the reasons for this are likely highly complex and not as straight forward as we may imagine. So, if we say 10% of all intelligent life throw up a species that is capable of developing technology (not that same thing as actually doing it), it gives us a start point.
- Of those species that are capable of technology, how many actually become a technical society? Modern humans have existed for about 300,000 years, but our technical development has only happened in the last 3000 years, about 1% of our time on Earth, so the development of technology is perhaps not overtly common, so if we say only 10% of capable species actually develop technology (until we have more statistics of course), it gives us a figure to work with.
Now we have some data points to work with.
So, with this we can do a tweak of the classic Drake Equation.
N= Ns*Sp*Pn*PHZ*PL*Lc*Lci*LTc*LTd
Where:
Ns = Total number of stars in the galaxy considered to be hosts for metal rich planets.
Sp = Total number of those stars with planets.
Pn = Averaged number of planets in planetary systems.
PHZ = The percentage of planets in the habitable zone of the star.
PL = The percentage of those planets in the habitable zone that develop life.
Lc = The percentage of life bearing planets where complex life evolves.
Lci = The percentage of those worlds with complex life that throws up intelligence in species.
LTc = The percentage of intelligence that is capable of developing technology.
LTd = The percentage of technical capable species that actually develop an advanced and industrial society.
Thus, we put in data.
N = 88,750,000,000*98%*7*20%*33%*50%*100%*10%*10% = 200,912,250 planets upon which a technical society may exist.
Now we know the volume of space that these societies are most likely to exist in – 308,505,125,000,0003 so we end up with a technical society in an averaged volume of 1,535,521.72653 Light years, based on our calculations above that would average a span of space some 5,137.1443 light years in diameter.
That shows that whilst the galaxy may be teeming with life, potentially over 200 million advanced societies, it also means that the nearest may be a considerable distance from us – space is huge, very huge, and so communications across these distances may actually be impractical in the lifetime of a given civilisation, depending on how long that may be.
If we look at human history, Luke Kemp (University of Cambridge) calculated, in 2019, that the average lifespan for a given civilisation is a mere 336 years, see below.
Now, if we assume a civilisation lives longer the greater the technology, then we could expect a civilisation to exist for perhaps a few thousand years, after all, no matter how technological you become, there are certain natural events that may be beyond the control of technology.
Many civilisations on Earth were taken out by natural disasters, volcanoes, tsunami, earthquakes or a combination of these, but it is also reasonable to expect other natural disasters to befall a civilisation. Minor planets (asteroids and associated fragments) can and do impact planets, it is not difficult to understand that the nascent technological society could be wiped out or sent backwards quite severely by the impact of a “space rock”. Further, bacterial and viral species change continually, the recent pandemic for SARS-COV-2 (COVID-19) is a prime example of how a pathogen can come out of the blue and have a huge and devastating impact on a civilisation. The Influenza Pandemic (1918-1920), commonly called “Spanish Flu” (Incidentally, it is only called that because the Spanish were the first to publicly speak about the infection, Allied and Axis powers did not publicly acknowledge the pandemic until after the cessation of hostilities because it was deemed too sensitive – it arrived from Asia and not Spain) infected about 500 million humans (about 1/3rd of the then human population) killed upwards of 50 million of them.
The Black Death, thought to be Bubonic Plague, killed an estimated 200 million people in Europe in the 14th century and that undoubtably had a major impact on the history of the continent and the wider human race.
An uncontrollable outbreak of a haemorrhagic virus, such as Ebola or Marburg, could wipe out a significant proportion of the human race, and to push our history back hundreds, or even thousands of years, if it wiped out most of the Industrialised world, where living in close proximity makes humanity more vulnerable, then that means our civilisation had died and would need to start afresh – but this would take considerable time.
It is naïve to assume that civilisations on other worlds would not face similar threats to their existence, and it is likely some species on other worlds may be wiped out by natural disasters, perhaps not directly, but by attrition. Humanity faced just such a bottleneck some 74,000 years ago when the volcano that we now call Lake Toba erupted in a super eruption, changing the climate of Earth for perhaps hundreds of years – the archaeological record indicates that the human race was reduced to, at most, about 10,000 members of the species immediately after this eruption, and we took thousands of years to recover.
It is not inconceivable (though unlikely) that the dinosaur group evolved a technically advanced species that built cities and had what we would recognise as technology – but sadly, their technology did not, and could not, prevent the impact of a 10km asteroid in what we call The Yucatan Peninsula in Mexico – wiping out 73% of all species alive on Earth at that time – this was 66 million years ago – there would be no evidence of their civilisation we could reasonably expect to find had they existed before you all get excited by that thought.
What all this means is that whilst there is likely to be hundreds of millions of technically advanced civilisations littering the galaxy with their robot probes and other detritus, they are likely hundreds to thousands of light years away from us and we simply have missed any signal they may have allowed to drift out into the cosmos, or they simply have not sent one within the timescale we have been able to receive it – they would need to be closer than about 60 light years for a realistic identification of an artificial signal – and as their technology evolves, they likely use directed communications rather than spray and pray broadcast signals – which would result in little leakage.
There is also the technology barrier – if an aircraft from today went back in time (lets ignore how that happened) and tried to communicate with ground controllers in the 1930s/40s then they would be unable to speak to each other because the carrier signals, the broadcast signal and equipment would be incompatible – we likely would have the same issue with an alien intelligence – we may be able to detect that the signal was not a natural one, but it is unlikely we would be able to decipher it – especially in the way they show in films!
So where does all this leave us when we are looking for intelligent life out there in the void?
I would suggest we need to narrow our searches to the stars that are most likely to host planets with complex life, and I would further suggest this should be stars in the mass range from 0.5 to 1.5 times that of the Sun, simply because this mass range offers sufficient stable, metal rich and long lived stars, those living in excess of 3 billion years, which is likely the minimum length of time a star needs to be on the main sequence for a planet to have a very high chance of developing complex life and also technological capable species.
Our technology and understanding are advancing all the time, if we find life in one or more ecosystems on other worlds in our solar system, then our view of life’s origins needs to be reviewed and amended, but if we do not, that will be a serious dent in our thought process on life’s origins.